- MOE Key Laboratory of Cell Activities and Stress Adaptations, School of Life Sciences, Lanzhou University, Lanzhou, China
Maintaining lipid membrane integrity is an essential aspect of plant tolerance to high temperature. P4-type ATPases are responsible for flipping and stabilizing asymmetric phospholipids in membrane systems, though their functions in stress tolerance are not entirely clear. Aminophospholipid ATPase6 (ALA6) is a member of the P4-type ATPase family, which has 12 members in Arabidopsis thaliana. Here, we show that a loss-of-function mutant of ALA6 (ala6) exhibits clear sensitivity to heat stress, including both basal and acquired thermotolerance treatments. Overexpression of ALA6 improves seedling resistance to heat stress, while mutated ALA6 transgenic plants, in which the conserved functional site of the ALA family has a point mutation, are still susceptible to heat stress like ala6 loss-of-function mutant. In addition, ala6 displays higher ion-leakage during heat treatment, suggesting that the lipid flippase activity of ALA6 plays a vital role in heat stress responses. Transcriptome analysis reveals differences in gene expression between ala6 and wild-type plants with or without heat stress. The differentially expressed genes are involved primarily in the physiological processes of stress response, cellular compartment maintenance, macromolecule stability and energy production. Our results suggest that ALA6 is crucial for the stability of membrane when plants suffer from high temperature stress.
Introduction
The phospholipid bilayer of cell membranes is asymmetrical, as the abundance of various lipid species on one side differs from that on the other. Within the membrane leaflets, the positions of the phospholipids are not fixed. Phospholipids perform multiple intramolecular motions, including rotation and lateral diffusion, and can also flip-flop between the two leaflets (Pomorski and Menon, 2016). The ambient temperature has a direct effect on the rate and frequency of these movements, eventually altering membrane fluidity. Thus, maintenance of the thermodynamic balance of the membrane is vital for cell function, especially during abrupt temperature changes (Murata and Los, 1997; Vigh et al., 1998, 2007; Horváth et al., 2012).
Studies have revealed that lipid asymmetry via flip-flopping across membrane leaflets is involved in numerous functions of the membrane system, including formation and maintenance of cell shape; vesicle budding; membrane trafficking, impermeability, and rigidity; extra- and intracellular signaling; fertilization; apoptosis; and membrane-coupling protein regulation (Puts and Holthuis, 2009; Andersen et al., 2016). Generally, the chemical traits of phospholipids dictate that transbilayer exchange of polar lipids should occur slowly and rarely. In fact, lipid translocation across the two leaflets is greatly assisted by flippases/floppases and/or scramblases. The membrane proteins that carry out these lipid translocation processes are classified into two categories based on whether the lipid transportation across the bilayer is driven by ATP (Pomorski and Menon, 2006, 2016). P-type ATPases are found in all kingdoms of life and possess a conserved aspartic acid residue within the P-type motif DKTGT that mediates reversible phosphorylation and conformational changes during substrate transport (Pedersen and Carafoli, 1987). Based on substrate specificity and sequence characteristics, P-type ATPases are divided into five subclasses, P1- through P5-type ATPases (Palmgren and Axelsen, 1998). P4-ATPases are flippases, which utilize the energy from ATP hydrolysis to transport specific phospholipids from the exoplasmic to the cytoplasmic face of the membrane against their concentration gradient (Coleman et al., 2009, 2013; Zhou and Graham, 2009; Lopez-Marques et al., 2014). These proteins are unique in that they flip “giant” phospholipids across membranes and are found only in eukaryotic cells (Paulusma and Elferink, 2010; Van Der Mark et al., 2013; Andersen et al., 2016). The first five P4-ATPases (Neo1p, Drs2p, Dnf1p, Dnf2p, and Dnf3p) were found in yeast, and at least 14 such proteins (ATP8A1 to ATP11C) occur in mammals (Paulusma and Elferink, 2010; Sebastian et al., 2012; Van Der Mark et al., 2013; Andersen et al., 2016). Most P4-ATPases have unique lipid specificities and subcellular localization. For example, Drs2p/ATP8A1 carries out PS and PE translocation in the late secretory pathway, while ATP8B2 and ATP10A were found to mediate PC asymmetry specifically (Ding et al., 2000; Alder-Baerens et al., 2006; Paterson et al., 2006; Xu et al., 2009; Zhou and Graham, 2009). Flippases with a relatively wide range of phospholipid specificities also exist, such as Dnf1p and Dnf2p, which can transport PC, lysophospholipids and synthetic alkylphospholipids in plasma membranes (Pomorski et al., 2003; Riekhof and Voelker, 2006; Baldridge et al., 2013).
Unlike those in yeast and mammals, the P4-ATPase in the plant Arabidopsis thaliana was identified much later and its function remains unclear. There are12 P4-ATPase proteins, ALA1 through ALA12 (Aminophospholipid ATPase subfamily) in Arabidopsis (Axelsen and Palmgren, 1998). Recent reports indicate that ALA2 internalizes PS in the endosomal system, whereas ALA3, localized in the Golgi apparatus, carries out flipping of a broad range of lipids, including PS, PE and PC (Poulsen et al., 2008; López-Marqués et al., 2010). ALA10 is located in the plasma membrane and internalizes various phospholipids, including lysoPC (Poulsen et al., 2015). With respect to biological function, ALA1 may play an important role in chilling tolerance (Gomès et al., 2000), while ALA2 may function with ALA1 in antiviral defense (Guo et al., 2017). ALA3 is involved in secretory processes of the Golgi apparatus at the root tip to regulate root growth (Poulsen et al., 2008). ALA6 and ALA7 are crucial for pollen fitness (McDowell et al., 2015). In addition, ALA10 has been found to function in leaf and root development, as well as in stomatal control (Poulsen et al., 2015; Botella et al., 2016). Some reports have also indicated that the physiological functions of several plant P4-ATPases can be affected by changes in temperature (Gomès et al., 2000; McDowell et al., 2013, 2015; Botella et al., 2016). Nevertheless, the ways in which these flippases respond to some types of temperature stresses remain unclear.
In the present study, our results suggest that seedlings of a loss-of-function mutant of ALA6 (ala6) grow normally at standard temperatures, while they wither, turn yellow and eventually die under basal and acquired thermotolerance treatments. Overexpression of ALA6 can improve plant tolerance of high temperature, and ALA6 point-mutated transgenic plants lacking a conserved aspartic acid residue are still sensitive to heat stress. In addition, the results of a transcriptome analysis and observation of increased ion-leakage and Chl b/a ratios in ala6 plants during heat treatment further suggest that ALA6 may protect plant cells from heat stress via the maintenance of membrane stability and integrity.
Materials and Methods
T-DNA Insertion Mutants
The Arabidopsis thaliana plants used here were in the Col-0 background. The T-DNA insertion mutant used in this experiment was ala6 (SALK_150173), obtained from the Arabidopsis Biological Resource Center (Ohio State University1) with homozygous progeny determined via PCR screening (Alonso et al., 2003). The T-DNA insertion site is shown in Figure 2A, and all PCR primer sequences are given in Supplementary Table S1.
Plant Growth Conditions
Seeds were surface-sterilized in 20% bleach for 12–15 min, rinsed five times with sterile water, and sown on ½ MS medium containing ½ Murashige and Skoog salts (MS; PhytoTech, Lenexa, KS, United States), 1% (w/v) sucrose, and 0.8% (w/v) agar. Plates were incubated at 4°C for 3 days in the dark and then transferred to a growth chamber at 21 ± 2°C with long days (16-h light/8-h dark cycles). The growth chamber was illuminated with white light at ∼110 μmol m-2 s-1.
Gene Cloning and Transgenic Line Creation
Full-length cDNAs of ALA6 and ALA6 with a point mutation in the N-terminal aspartic acid at position 426 were amplified via PCR. These cDNA fragments were cloned into the pBIB binary vectors driven by the ALA6 gene promoter. Four-week-old wild-type (WT) Col-0 and ala6 mutant plants were transformed with A. tumefaciens (strain GV3101) using the floral dip method as described by Clough and Bent (1998). Homozygous transgenic plants were isolated on Basta. Four transgenic Arabidopsis lines were generated: complementary and overexpressing lines containing ALA6-autologous-promoter-ALA6, a tissue localization line containing the GUS gene driven by the ALA6-autologous-promoter, and a line expressing a point mutation in ALA6 driven by ALA6 gene promoter. The point mutation was an A-to-C conversion at base 1277 relative to the start codon of the full-length ALA6 cDNA (Supplementary Figure S1). All PCR primer sequences and vectors are shown in Supplementary Table S1.
Basal and Acquired Thermotolerance Treatments
Thermotolerance assays were performed on 14-day-old seedlings as previously described (Larkindale and Vierling, 2008; Mittler et al., 2012). The basal heat treatment consisted of incubation at 43.5°C for 45, 60, or 90 min. The acquired thermotolerance treatment consisted of incubation at 37°C for 1.5 h followed by recovery at 22°C for 2 h and then by heat shock at 43.5°C for 1, 1.5, or 2 h. Plants were then moved to a growth chamber for 30, 36, or 72 h prior to sample preparation, measurement or photography. All assays were repeated at least in triplicate.
Determination of Electrolyte Leakage
Fourteen-day-old seedlings were subjected to thermotolerance treatments and allowed to recover for 36 h. EL was determined as described by Sairam and Srivastava (2002). The sample (0.5 g) was placed in a 15-ml tube with 10 ml deionized water and incubated at 25°C for 2 h, and the conductivity in the solution was measured using a conductometer (R1). Samples were then heated for 15 min in a boiling water bath, and conductivity was measured again after the samples cooled to 25°C (R2). EL was calculated as the ratio of the initial conductivity to the conductivity after heating in boiling water (EL (%) = (R1/R2) × 100%). All assays were repeated at least in triplicate.
Measurement of Chlorophyll Contents
Samples were collected from 50 to 100 mg leaves for chlorophyll extraction. Chlorophyll contents were measured using 95% ethanol as described previously (Woo et al., 2001). Leaf samples of 50–100 mg were placed in a test tube and pulverized in liquid nitrogen. The freeze-dried powder was incubated with 1 ml 95% ethanol in the dark at room temperature for 2–3 h. After dilution with solvent to 1.5 ml, the mixture was centrifuged at 13400 × g for 5 min. One milliliter of the supernatant was diluted 2- to 10-fold with 95% ethanol (OD value = 0.1–0.6), and the chlorophyll level was determined by a spectrophotometer at 665 and 649 nm. All steps above were performed at room temperature (25°C). The assay was repeated at least in triplicate. Chlorophyll contents were calculated using the following formulae: Chl a = 13.7 × OD665 - 5.76 × OD649, Chl b = 25.8 × OD665 - 7.6 × OD649, and Chl a + b = 6.10 ×OD665 + 20.04 ×OD649. Chl a is the chlorophyll a content, Chl b is the chlorophyll b content, Chl a+b is the total chlorophyll content, and OD is the absorbance.
Semi-Quantitative RT-PCR
Fourteen-day-old WT and ala6 seedlings grown on MS agar plates were collected, placed in tubes, and stored in liquid nitrogen. Total RNA was isolated using the MiniBEST Plant RNA Extraction Kit (Takara Biotechnology Co., Ltd., China). First-strand cDNA synthesis reaction was performed as Takara manual using Reverse Transcriptase M-MLV (RNase H-) (Takara Biotechnology Co., Ltd., China). PCR was carried out via a TP350 thermal cycler (Takara Bio Inc., Otsu, Japan) with the following program: 3 min at 94°C (1 cycle); 30 s at 94°C, 30 s at 53∼60°C and 30 s∼1.5 min at 72°C (28/32 cycles); and 5 min at 72°C (1 cycle). Each PCR reaction was replicated for three times. ACTIN2 was selected as a reference gene (see Supplementary Table S1 for primer sequences).
Quantitative PCR
Fourteen-day-old WT, ala6 and COM seedlings grown on MS agar plates were treated at 43.5°C for 45 min and recovered for 30 h, then collected in liquid nitrogen. Total RNA was reverse-transcribed into cDNA by the methods above. qPCR was carried out using a Stratagene MX3005P Real-Time System (Agilent, United States) with SYBR Premix Ex Taq (Takara Biotechnology Co., Ltd., China) as manufacturer’s instructions. Program for the reaction was as follows: 95°C for 30 s, 40 cycles of 95°C for 5 s, 60°C for 30 s. Melt curves (0.5°C increments in a 60–95°C range) were performed for each gene to assess the sample for non-specific targets and primer dimers. UBQ11 was used as an internal control to normalize the expression of the target gene. A list of the primers used in these experiments is found in Supplementary Table S1. The ΔΔCt method was used to analyze relative transcript abundance (Rieu and Powers, 2009). The results were based on three independent experiments.
GUS Staining
To identify the tissue-specific localization of ALA6, independent plants from each GUS-transgenic line were selected and various tissues were stained (Jefferson et al., 1987). Samples were incubated in a solution of 2 mM 5-bromo-4-chloro-3-indolyl-β-D-glucuronide (X-gluc), 3mM K3(Fe(CN)6), 3M K4(Fe(CN)6), 0.2% Triton-X-100, and 50 mM KH2PO4/K2HPO4(pH 7.2) at 37°C in the dark for overnight. Samples were then rinsed in 95% ethanol to remove chlorophyll. The samples were observed and photographed via anatomic microscope (SMZ-168, Motic Inc.).
Transcriptome Analysis
Fourteen-day-old WT and ala6 seedlings grown on MS agar plates were incubated at 43.5°C for 45 min and allowed to recover for 30 h. Gene expression analysis was performed by the Novogene Corporation, Beijing, China. RNA libraries were sequenced on a HiSeq 2500 instrument. TopHat version 2.0.122 was used for mapping against the Arabidopsis genome3. HTSeq v0.6.1 was used to determine the read numbers for each gene. Based on the length of each gene and the corresponding number of reads, the FPKM value of each gene was calculated. Three independent experiments were conducted, and only genes having consistent expression changes in the three microarray assays were reported. DEG analysis was performed using the DESeq R package (1.18.0) with an adjusted P-value (<0.05). In addition, Gene Ontology (GO) enrichment analysis of DEGs was carried out using the GOseq R package, in which results were corrected for gene length bias. GO terms with corrected P-values less than 0.05 were considered to be significantly enriched DEGs.
Statistical Analysis
Each experiment was repeated at least three times. Data were analyzed using one-way ANOVA with Turkey’s multiple-comparison test under a 0.05 confidence coefficient.
Results
Tissue Expression Pattern of ALA6
To analyze the tissue specificity of ALA6, its promoter region was fused to a GUS reporter gene and stably expressed in Arabidopsis plants. After staining, GUS signals were found throughout the young seedling except in the root tip, with particularly strong expression at the junction between the root and hypocotyl (Figure 1A(a)). In the leaf, the signal penetrated the veins and was especially strong in the leaf margin (Figure 1A(b)). In the flower and silique, staining was strong in the stigma, anther and base and tip of the silique; the signal was also particularly intense in pollen (Figure 1A(c,d)). Gene expressed in different tissues via semi RT-PCR analysis also showed that ALA6 was expressed in reproductive organs more than that in vegetative tissues (Figure 1B). The expression of ALA6 in numerous tissues suggests that ALA6 may be involved in multiple physiological activities in the plant.
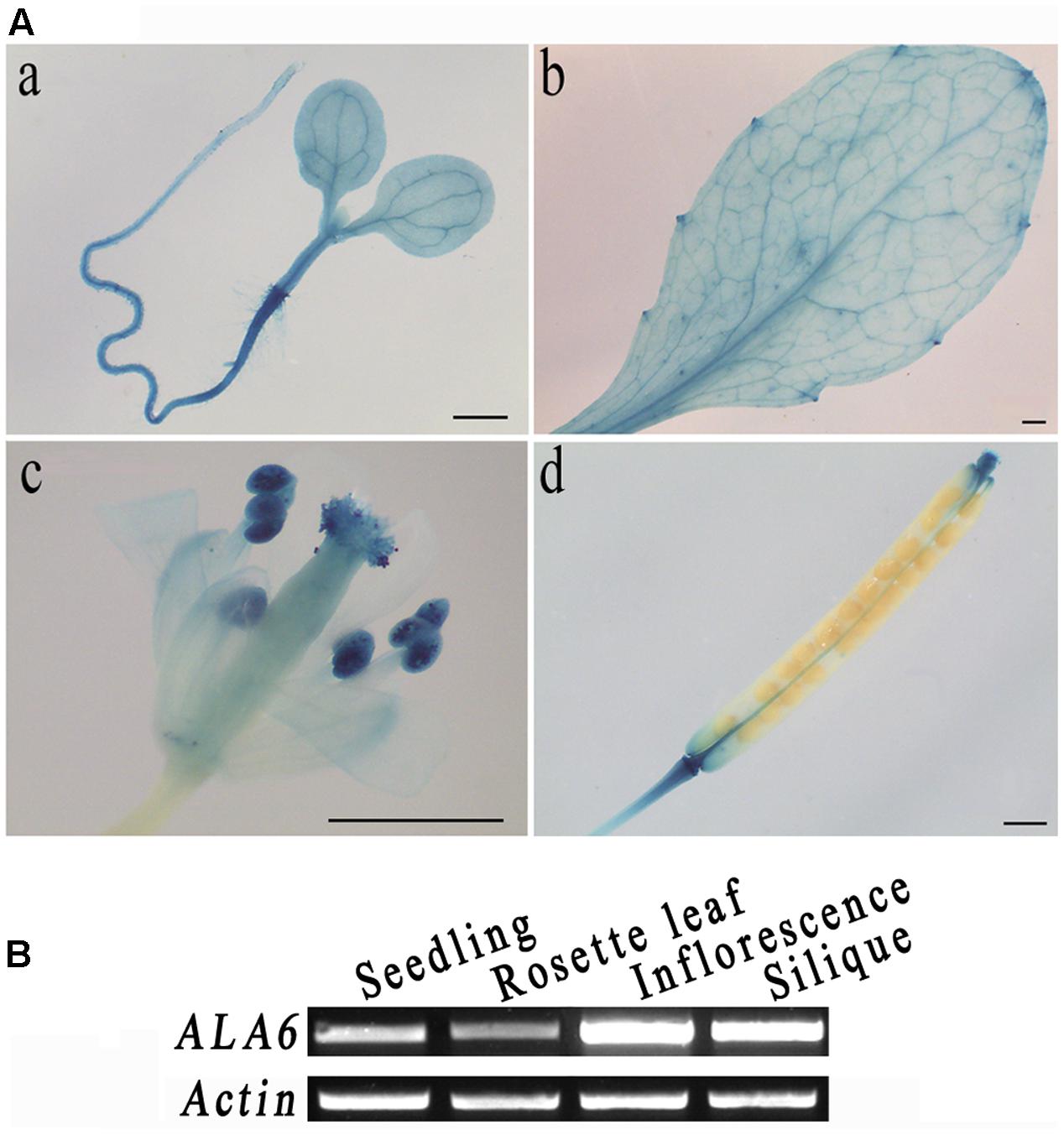
FIGURE 1. Tissue-specific expression of ALA6. (A) a, 5-day-old seedling. b, rosette leaf. c, flower, stamen, and pistil. d, silique. (B) ALA6 expression in various tissues via RT-PCR assay.
Loss of Function of ALA6 Confers Hypersensitivity to Heat Stress in Arabidopsis Seedlings
To determine the function of ALA6, a T-DNA insertion mutant was obtained from the SALK collection (SALK_150173). The T-DNA was inserted into the first exon of ALA6 (Figure 2A). The homozygous F2 progeny of ala6 plants were screened (Figure 2B) and tested to determine ALA6 expression levels, and it was found that the expression of ALA6 in this T-DNA insertion line was completely suppressed (Figure 2C).
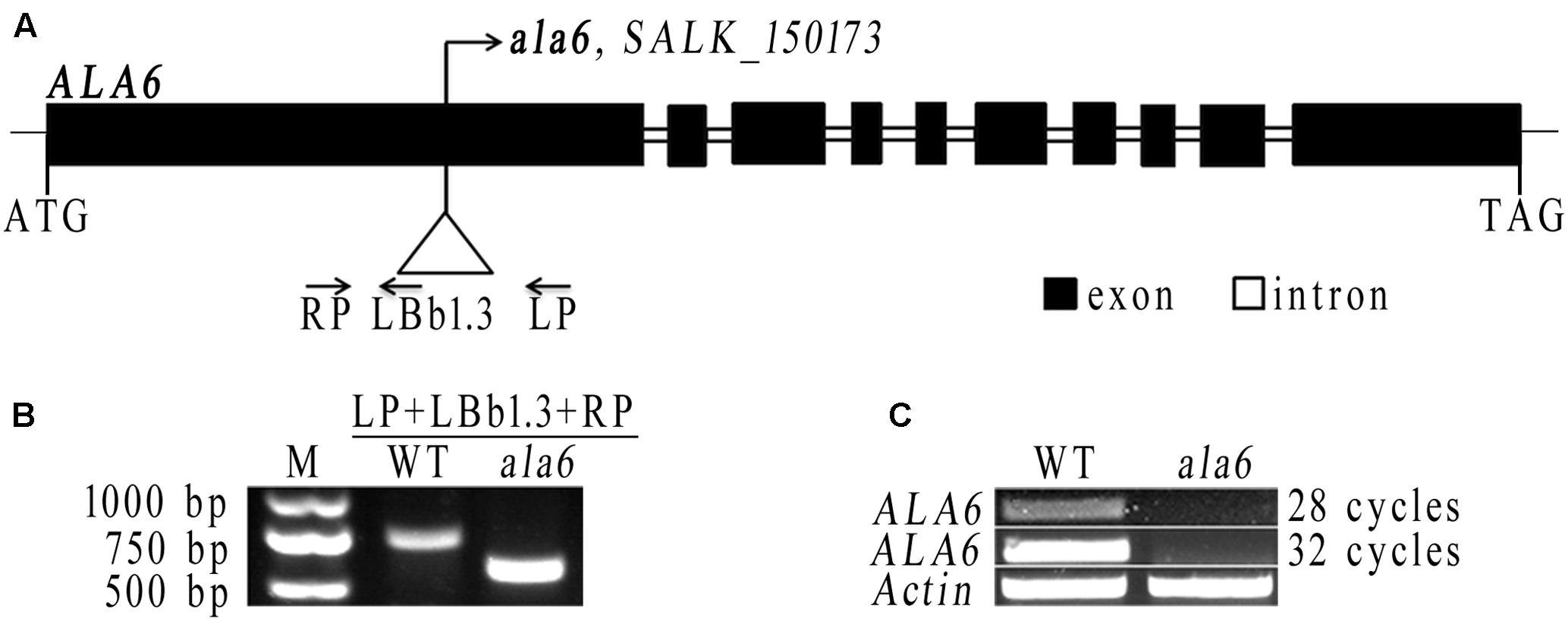
FIGURE 2. The T-DNA insertion line of ALA6. (A) Diagram of the ALA6 gene and the T-DNA insertion site. (B) Identification of homozygous ala6 mutants, using the primers shown in (A). (C) Semi-quantitative analysis demonstrates that ALA6 is knocked-out in the ala6 mutant. 28 cycles and 32 cycles are cycles in PCR amplification.
As ALA6 has been predicted to function in response to high temperature stress, two different experiments were performed to determine the heat-sensitive phenotype of ala6 at a lethal temperature of 43.5°C (Figure 3B). As shown in Figure 3A, the first treatment consisted of incubation at high temperature for different lengths of time (45, 60, or 90 min) followed by recovery for 3 days. Compared to WT, ala6 was extremely sensitive to heat stress. In the 45-min treatment, some ala6 seedlings began to wilt, with leaves shrinking, curling, and yellowing. Seedlings also grew more slowly or died (Figure 3A(b)). After longer heat-treatments, the lethal effect of the ala6 mutation was more obvious (Figure 3A(c,d)).
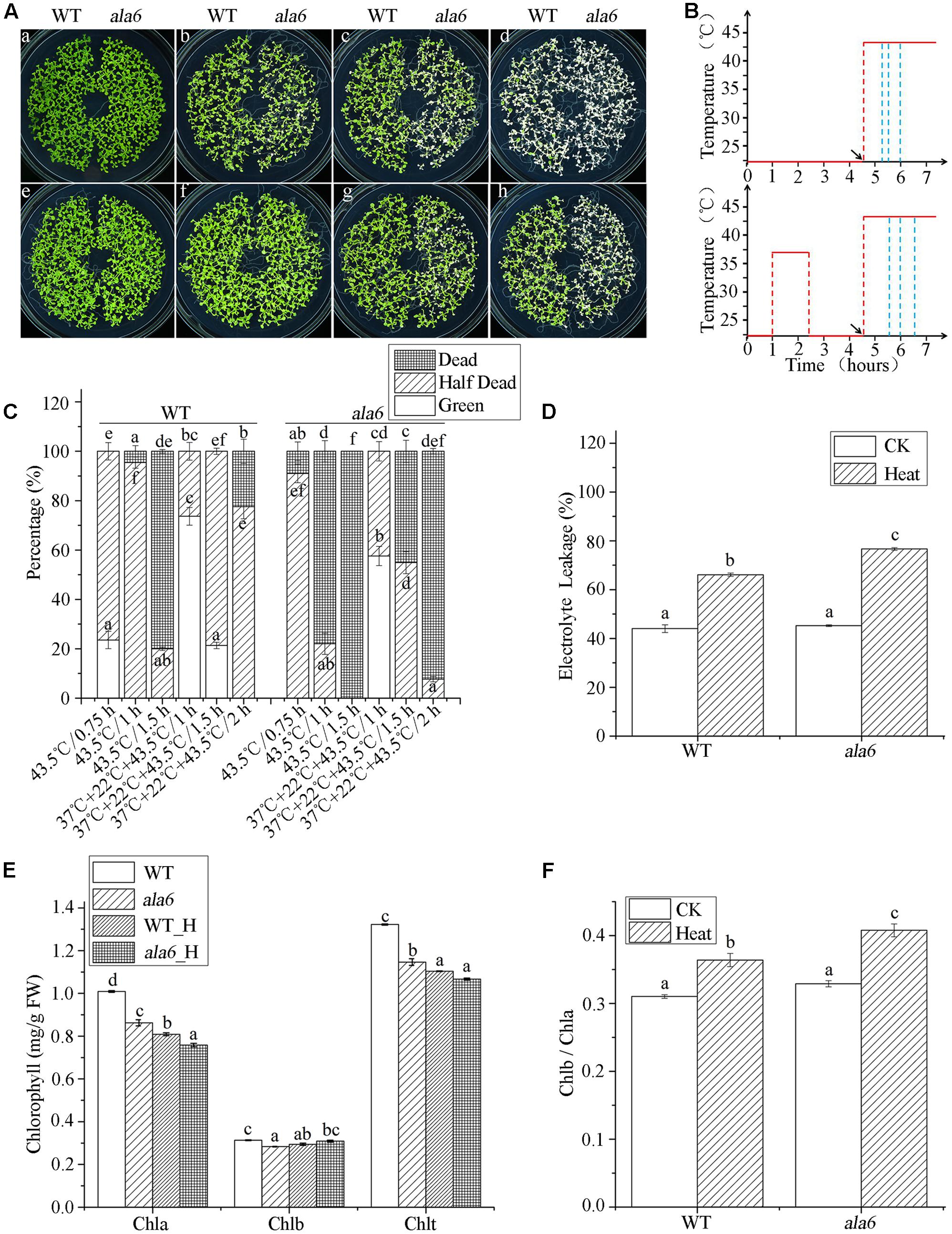
FIGURE 3. ala6 is hypersensitive to heat stress. (A) a and e are 14-day-old WT and ala6 seedlings without thermal treatment; b was incubated at 43.5°C for 45 min; c and f were incubated at 43.5°C for 1 h received prior heat acclimation at 37°C for 1.5 h; d and g received the same treatments as c and f, respectively, but the heat shock time was 1.5 h; h received acclimation at 37°C prior to heat treatment for 2 h. (B) Schematic diagram for heat treatment corresponding to (A); from top to bottom, linear graphs represent basal thermotolerance (no acclimation) and acquired thermotolerance (acclimation). Red lines indicate the temperatures and blue lines indicate different treatment times. Arrows illustrate the times of the heat shock at 43.5°C. (C) The percentage of seedlings, including dead, half-dead and alive. The six columns correspond to b, c, d, f, g, and h in (A) are calculated from three independent experiments (average results ±SE). Columns with different letters have significantly different values (P < 0.05). (D) Electrolyte leakage (%) of 14-day-old seedlings treated at 43.5°C for 45 min and allowed to recover for 36 h. (E) Chlorophyll contents (mg/g) of seedlings as in (D). WT_H, wild type treated with heat stress; ala6_H, ala6 treated with heat stress; Chla, chlorophyll a; Chlb, chlorophyll b; Chlt, total chlorophyll. (F) Chlb/Chla ratio of (E). Results are calculated from three independent experiments (average results ±SE). Columns with different letters have significantly different values (P < 0.05).
The second heat treatment consisted of exposure to a constant, non-lethal temperature (37°C) to allow acclimation, followed by incubation at an optimum temperature (22°C) prior to exposure to a lethal temperature (43.5°C) (Figure 3B). As shown in Figure 3A(g,h), ala6 remained sensitive to high temperature. Compared with the basal heat-treatment, both WT and ala6 plants showed improved tolerance to high temperature after a brief period of acclimation. With preliminary heat acclimation, most WT seedlings survived, and approximately 50% of ala6 seedlings turned yellow after 90 min at 43.5°C (Figures 3A(g),C). Without previous heat acclimation, only a few WT seedlings showed any viability, and all ala6 seedlings were dead after 90 min at 43.5°C (Figures 3A(d),C). These results indicated that plants exposed to non-lethal temperatures in advance of abrupt exposure to high temperature showed improved responses to heat stress. In addition, it appeared that ala6 was inherently heat-sensitive, showing susceptibility to heat treatment even after warm acclimation. ALA6 might play a crucial role in plant heat-tolerance.
Considering the conserved function of the ALA family in phospholipid transport, the membrane status of ala6 plants under hyperthermia was investigated. WT and mutant seedlings were grown on MS plates for 14 days and incubated at 43.5°C for 45 min, followed by a 36-h recovery under optimum conditions. After this treatment, the growth of all seedlings was affected. Seedlings were collected, and EL was quantified; the results were shown in Figure 3D. Heat treatment significantly increased the rate of EL in both WT and mutant plants. Leakage rates increase by 48.9 and 68.9% due to heat treatments in WT and ala6 seedlings, respectively. Notably, the membrane status of ala6 cells was altered more than that of WT cells, implying that ALA6 might protect plant cells from heat stress by regulating plasma membrane permeability and stability.
Changes in the contents of photosynthetic pigments (chlorophylls) and in Chl b/a ratios are useful indicators of stress and tolerance in plants (Zhang et al., 2008). We found that chlorophyll levels decreased during heat stress treatments in both WT and ala6 mutant plants, indicating possible heat-induced damage to the photosynthetic activity of the chloroplasts (Figure 3E). In addition, the chl b/a ratio increased dramatically in ala6 mutants after basal heat treatment, reaching a level 36.4% higher than in ala6 mutants without treatment, whereas the ratio in WT increased only 28.2% (Figure 3F). These results indicated that a lack of ALA6 might increase the injurious impact of heat stress on membranous organelles.
The Intrinsic Function of ALA6 Is Critical for Thermotolerance
To confirm the function of ala6 under high temperature, an exogenous ALA6 gene (normal or deficient in conserved gene function) was expressed in ala6 and WT plants. The complementation lines resulting from the former transformation were subjected to heat treatment, as shown in Figure 4, and it was found that the expression of ALA6 could rescue the heat-lethal phenotype of the loss-of-function mutant. The growth of the complementation lines resembled that of WT plants, even after heat treatment. In addition, the ALA6 overexpression lines exhibited greater vitality than WT plants (Figures 4A,C), showing that ALA6 was involved in intracellular responses to heat stress, and the heat-sensitive phenotype of ala6 was due to the loss of function of ALA6.
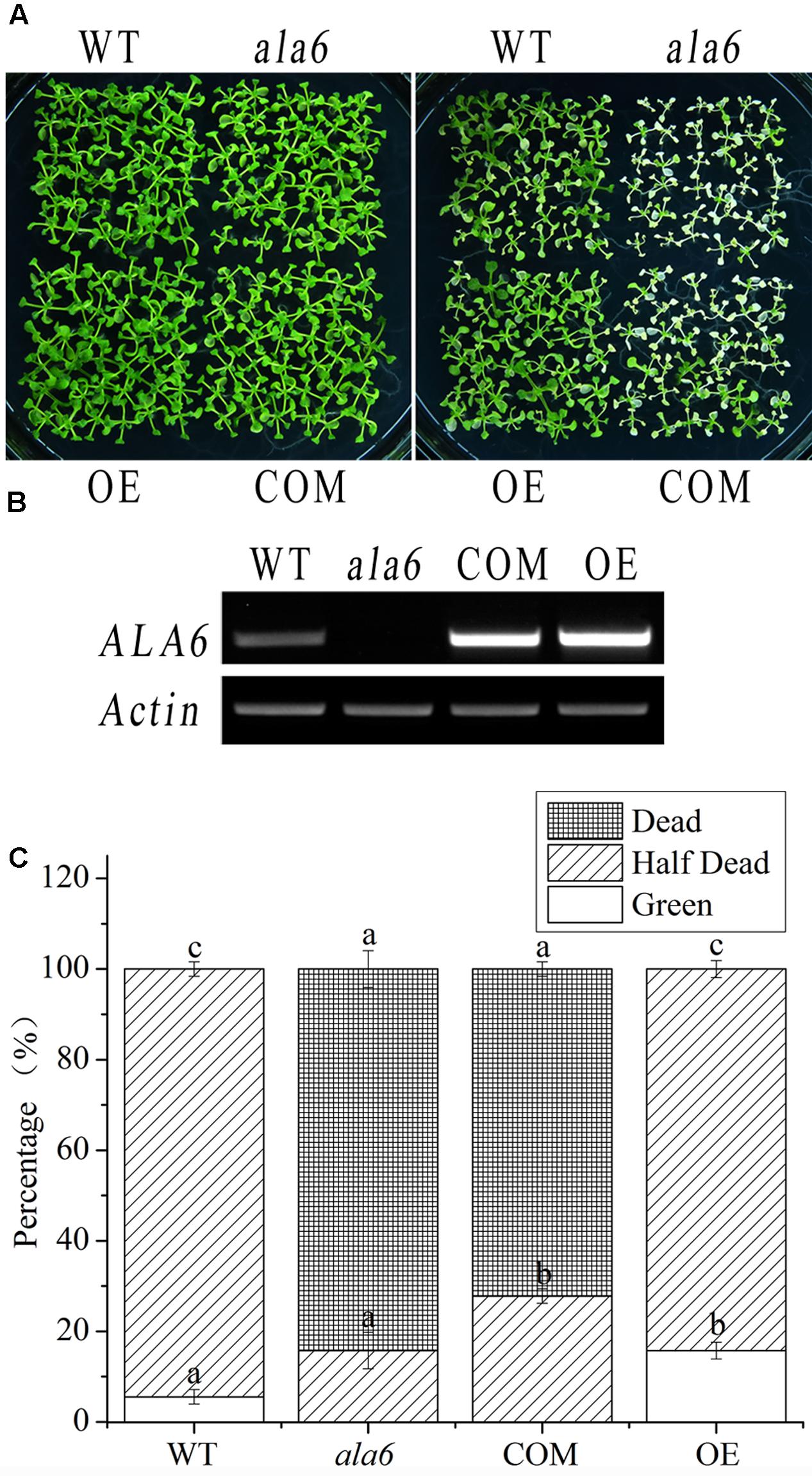
FIGURE 4. Complementation and overexpression lines of ALA6 under basal thermotolerance. (A) Phenotypes of complementation and overexpression lines under heat stress. WT, wild type; ala6, ALA6 T-DNA insertion line; COM, complementation line of ALA6; OE, overexpression line of ALA6. (B) RT-PCR analysis of ALA6 expression in complementary and overexpressed transgenic lines. (C) Survival rate of seedlings corresponding to (A). Results are calculated from three independent experiments (average results ±SE). Columns with different letters have significantly different values (P < 0.05).
P-type ATPases, including the ALA family in Arabidopsis, contain a motif that carries out phosphorylation during phospholipid transport; this motif contains an aspartic acid residue (Pedersen and Carafoli, 1987). To determine the relationship between the phospholipid transport activity of ALA6 and the heat stress response, transgenic lines containing a point mutation in the phosphorylation motif were generated. This mutation converted an A to a C in the codon encoding the conserved aspartic acid residue of ALA6. As expected, the transgenic lines showed a heat-hypersensitive phenotype like that of ala6 under high-temperature treatment (Figure 5A). This result further demonstrated that the native phospholipid transport activity of ALA6 was implicated in heat-tolerance, and the specific phenotype of ala6 was associated with a defect in its enzymatic activity. The levels of ALA6 transcript in these transgenic lines were measured and were significantly higher than those of the WT (Figures 4B, 5B). This observation was also supported by the survival rate shown in Figures 4C, 5C.
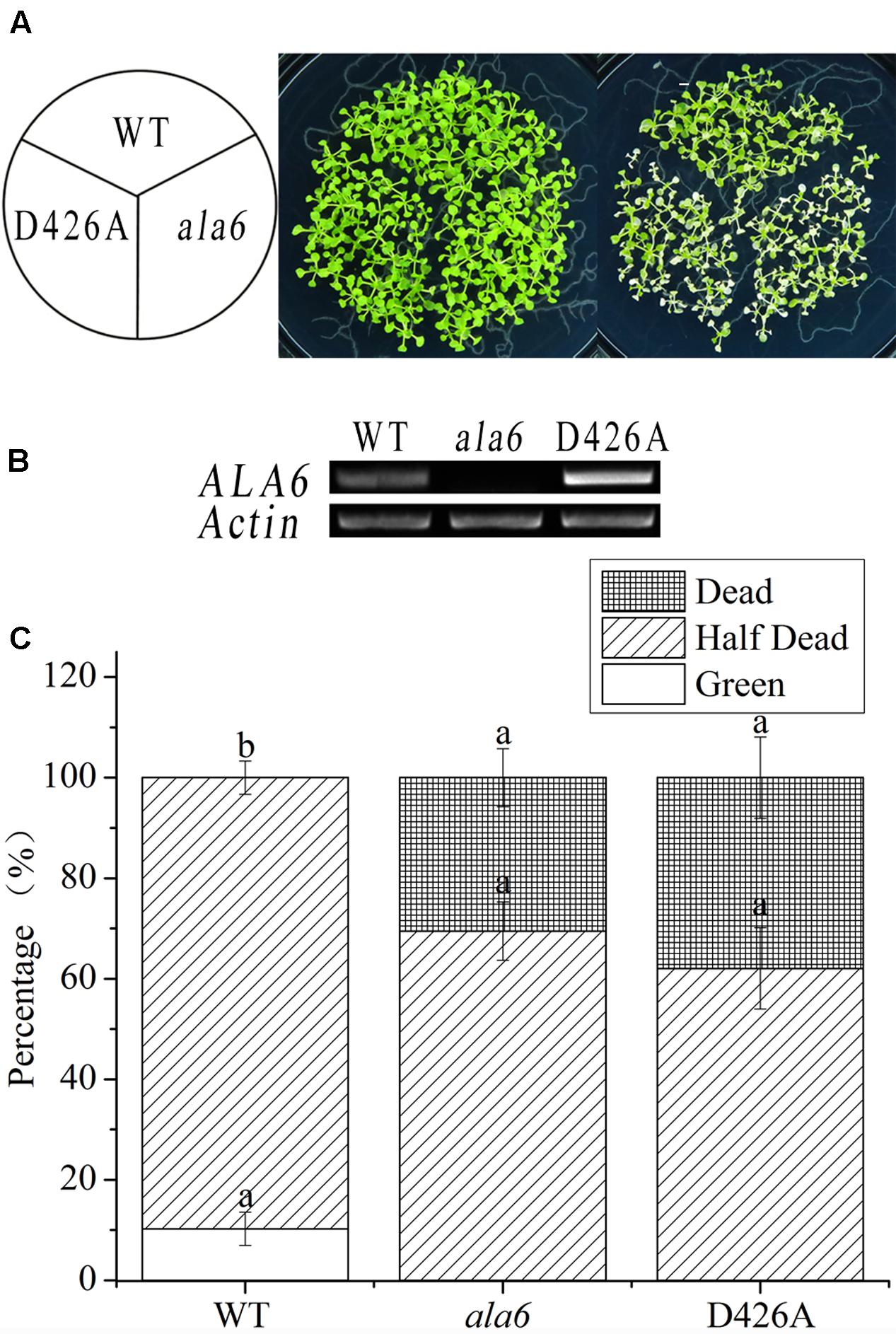
FIGURE 5. Point-mutant lines of ALA6 under basal thermotolerance. (A) Phenotype of point-mutant lines under heat stress. WT, wild type; ala6, ALA6 T-DNA insertion line; D426A, conserved aspartic acid-point mutant line of ALA6. (B) RT-PCR analysis of the expression of ALA6 in point-mutant transgenic lines. (C) Survival rate of seedlings corresponding to (A). Results are calculated from three independent experiments (average results ±SE). Columns with different letters contain significantly different values (P < 0.05).
Microarray Analysis Reveals ALA6-Related Genes
To investigate the underlying mechanism of heat sensitivity in ala6, we analyzed the RNA-seq data of WT and ala6 Arabidopsis samples. A total of 426 DEGs were found to be related to ALA6 (Figure 6A). Of these, 286 genes showed down-regulated and 140 showed up-regulated expression in ala6. All the transcripts listed here exhibited twofold or larger changes in abundance. These DEGs were enriched in GO terms containing genes responsive to endogenous stimuli, chemical or organic substances, and biotic or abiotic stresses (shown in Supplementary Table S2). The top 30 DEGs that were annotated as stress response elements include various transcription factors, membrane components and transporters (Table 1). These results indicated that ALA6 might be involved in multiple cellular responses.
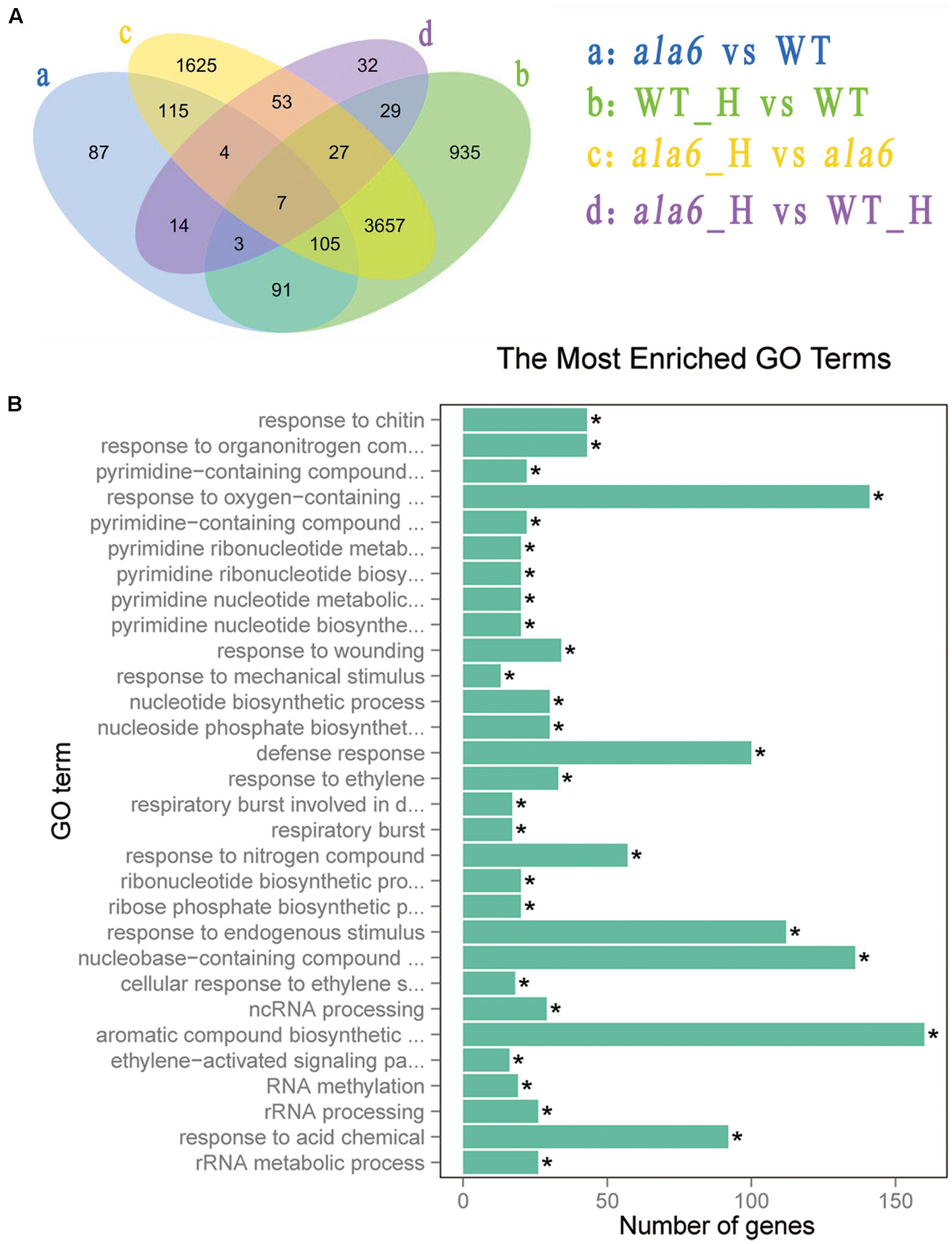
FIGURE 6. Differentially expressed genes after basal thermotolerance. (A) Venn diagram indicates DEGs. DEGs for a: ala6 vs. WT; b: WT with heat treatment vs. WT; c: ala6 with heat treatment vs. ala6; d: ala6 with heat treatment vs. WT with heat treatment. (B) GO enrichment for DEGs.
To identify genes related to ALA6 under heat stress, the RNA-seq data of Arabidopsis WT and ala6 samples with and without heat treatment were analyzed as described in the Section “Materials and Methods.” Quantitative PCR analyzing the expression of several DEGs in WT, ala6, ALA6 complementary transgenic lines with or without heat stress also confirmed the results from RNA-seq data above (Figure 7). Figure 6A showed the DEGs for ala6 vs. WT (a), WT with heat treatment vs. WT (b), ala6 with heat treatment vs. ala6 (c) and ala6 with heat treatment vs. WT with heat treatment (d). It was believed that genes related to ALA6 under heat treatment should be found in b but not in c (Figure 6A). A total of 1058 DEGs were identified and implicated in heat stress responses related to ALA6. The top 30 DEGs in Table 2 are involved in transcription factor activity, organelle components, and signal transduction and substrate transport. Of these genes, two transcription factors [BBX14 (AT1G68520) and ERF104 (AT5G61600)] had previously been implicated in stress response. And most of genes we screened were involved in photosynthesis-related activities (AT1G16720, AT4G12800, AT1G52870, AT2G30570, and AT1G06430) and transmembrane transport activity (AT5G49730, AT1G64720, AT5G46110, AT4G25570, and AT3G13062).
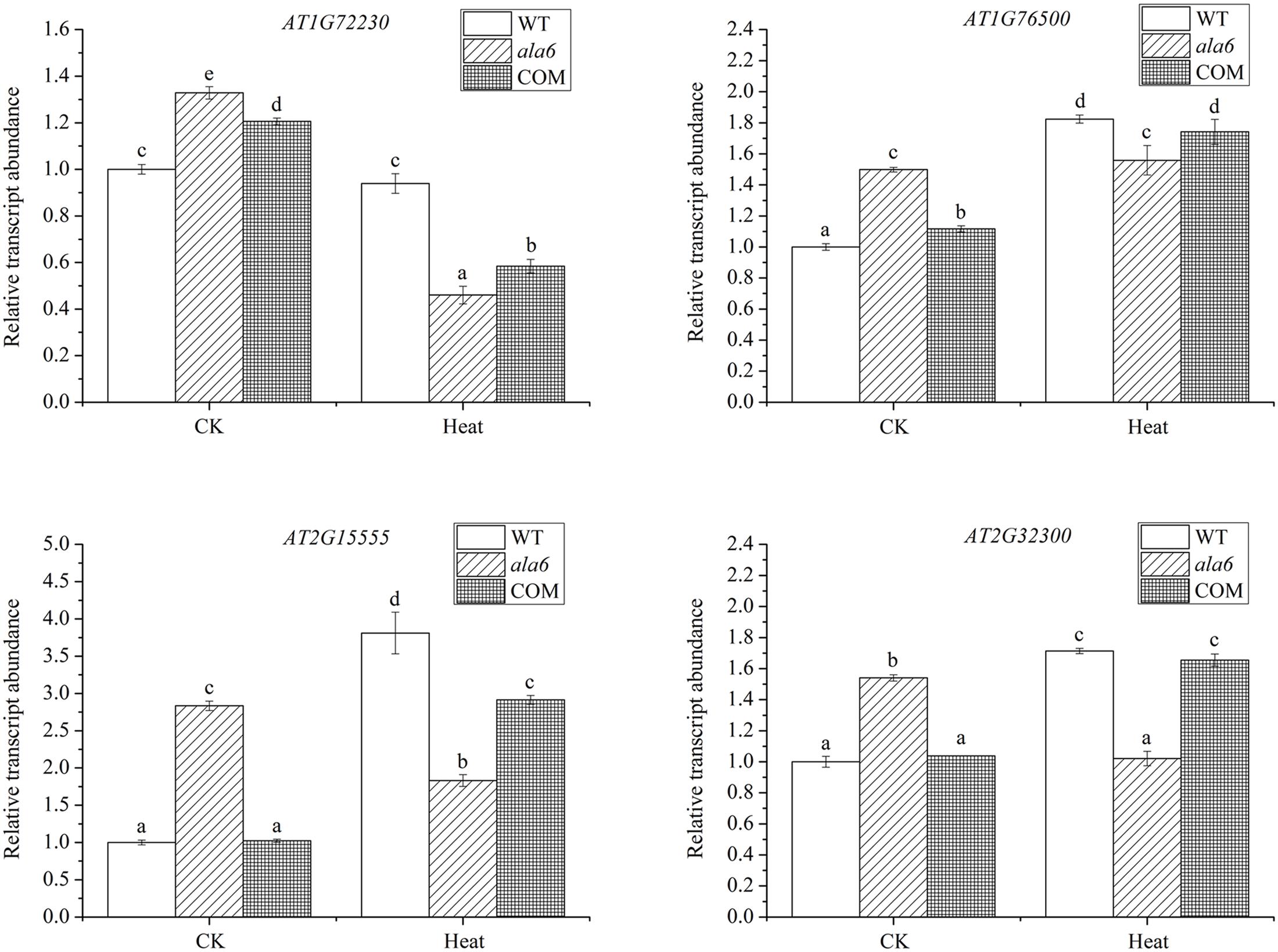
FIGURE 7. Relative transcript abundance of DEGs randomly selected in WT, ala6, and ALA6 complementary lines with or without heat stress. Results are calculated from three independent experiments (average results ±SE). Columns with different letters contain significantly different values (P < 0.05).
Differentially expressed genes from the basal heat treatment were subjected to GO enrichment analysis (Figure 6B). The genes associated with ALA6 under heat stress included 112 genes categorized as “response to endogenous stimulus” and 194 categorized as “response to stress.” Other categories of enriched genes include “response to oxygen-containing compound” (141 genes), “defense response” (100 genes), “response to acid chemical” (92 genes), and “aromatic compound biosynthetic process” (160 genes). These results indicated that ALA6 might have versatile effects on cellular responses triggered by heat stress.
Discussion
ALA6 Is Involved in Heat-Induced Cellular Responses via Maintenance of Membrane Stability
Temperature is a major environmental cue that has a rapid impact on cellular homeostasis, including both membrane stability and protein activity, and also has significant effects on plant development and growth. Because land plants are sessile, they are exposed to daily and seasonal temperature fluctuations (Saidi et al., 2011). To survive, plants evolved a series of mechanisms to respond to the ambient environment, including membrane-related stress signaling mechanisms for intact cell (Vigh et al., 2005, 2007). Stress-triggered changes in the lipid species of membranes could influence both the physical properties of the membrane and the distribution and activity of membrane proteins (Vigh et al., 2005). P4-type ATPases have recently been found to alter membrane lipid composition by transporting specific lipids, and some members of this protein family have been found to play crucial roles in stress responses in yeast and mammals (Axelsen and Palmgren, 2001; Sebastian et al., 2012). Among the 12 members of the P4-type ATPase family in Arabidopsis, ALA1 was first reported to be implicated in cold tolerance (Gomès et al., 2000). In addition, pollen tube development in an ala6/7 double mutant is described to be vulnerable under hot-day/cold-night temperature stress (McDowell et al., 2015). ALA3 has been demonstrated to participate in secretory processes of the Golgi apparatus, regulating root growth and reproductive development as well as tolerance to temperature stress (Poulsen et al., 2008; McDowell et al., 2013). Though ALA10 is responsible for root and leaf development independent of temperature, it can improve MGDG synthesis at low temperature (Poulsen et al., 2015; Botella et al., 2016). However, the functions of other members of this subclass have not been determined. In fact, the members of the ALA family that exhibit lipid substrate specificity have been shown to be involved in many physiological processes in a temperature-dependent manner. Considering the relatively close phylogenetic relationship among ALA family members 3–10 (Poulsen et al., 2015), we chose to investigate ALA6 and found that this protein is essential for responses to high temperature stress. As shown in Figure 2A, though a short-term acclimation can alleviate heat injury to seedlings, two heat treatments lead to the death of ala6 seedlings, whereas WT seedlings survive. In addition, the partially sterile siliques of ala6 plants under heat stress (data not shown) are comparable to the description given by McDowell et al. (2015). Since plants also suffer other stresses while acquiring thermotolerance via heat acclimation (Larkindale and Vierling, 2008; Mittler et al., 2012), this study shows that ALA6 can be implicated directly in heat stress-related intracellular responses rather than in other stresses. To determine whether the enzyme activity of phospholipid translocation is involved in the heat stress response, ALA6 with a point-mutation at a conserved functional site was analyzed and was confirmed not to rescue the heat-sensitive phenotype of an ala6 knockout mutant (Figure 5A). Taken together with the greater EL and higher Chl b/a ratio of ala6 mutants (Demidchik et al., 2014; Bi et al., 2016), this result implies that ALA6 might serve as a membrane stabilizer via altering membrane permeability, especially during heat stress.
ALA6 Can Induce Changes in the Expression of Heat Stress-Related Genes
Under high temperature, changes in lipid composition affect membrane dynamics and initiate the corresponding signal transduction pathways (Vigh et al., 1998). It has been reported that membrane fluidity can modulate the transcription of heat-inducible genes (Carratù et al., 1996; Horváth et al., 1998). To identify the downstream signaling triggered by ALA6, we analyzed gene expression in WT and ala6 plants with and without heat shock. DEGs between WT and ala6 Arabidopsis were shown to be involved in many functions. Among the 286 down-regulated genes are numerous genes related to stress and hormone responses, including heat, cold, salt, drought, oxidative, and pathogen stress, as well as ethylene, abscisic acid, JA and salicylic acid in various other processes. These responses are involved in the control of gene expression by transcription factors, such as the F-box protein family, the B-box zinc finger protein family, WRKYs and ERFs (Table 1). For instance, the expression of BBX14 (AT1G68520), a B-box-type zinc finger protein, was significantly lower in ala6 than in WT Arabidopsis. Although the function of BBX14 is still unknown, expression of its homolog, BBX18, has been confirmed to be induced by heat shock and to affect seed germination and seedling survival negatively by suppressing the expression of heat-responsive genes (Wang et al., 2013). Other members of this family are also involved in responses to various biotic or abiotic stresses (Gangappa and Botto, 2014), likely explaining why ala6 plants are sensitive to heat stress. In addition, it was found that 140 genes with up-regulated expression in ala6 plant are responsible for various biological processes such as phosphorylation, substrate transport and synthesis, and lipid metabolism, but most of these genes have an integral membrane component (Table 1 and Supplementary Table S2). Of these genes, only two fell within the top 30 DEGs with increased expression; these were SLAH3 (AT5G24030) and AGP17 (AT2G23130). The products of these genes both localize to the plasma membrane. SLAH3 is an anion channels involved in mediating ion homeostasis, while AGP17 is a glycosylphosphatidylinositol (GPI)-anchored protein involved in extracellular signal perception and interaction with proteins in lipid rafts (Gaspar et al., 2004; Zhang et al., 2016). The results imply that lack of ALA6 might not only lead to the alteration of membrane permeability and integrity but also to corresponding changes in membrane proteins.
Interestingly, the expression of many chloroplast-related genes in the top 30 DEGs are shown to be up-regulated in ala6, whose functions depend greatly on membrane stability (Table 2; Boudière et al., 2014). A HSP81-3 (HSP90.3, AT5G56010), which functions as a molecular chaperone, and AOC2 (AT3G25770), which has AOC activity, are among the most interesting genes we identified. The major functions of HSPs include preventing proteins from misfolding and disaggregation and protecting membranes that are exposed to high temperature (Banti et al., 2010). Proteins such as HSP20, HSP70, HSP90 and HSP101 have been shown to be components of the heat shock response and assist in thermotolerance by adjusting protein aggregation (Nieto-Sotelo et al., 2002; Cazalé et al., 2009; Fragkostefanakis et al., 2015; Yu et al., 2016). Thus, the decreased expression of HSP genes in an ALA6 loss-of-function mutant may also demonstrate that ALA6 plays an important role in heat-inducible signaling. Moreover, AOC2 catalyzes the biosynthesis of JA, for which a role in basal thermotolerance has been illustrated recently (Clarke et al., 2009; Stenzel et al., 2012). In conclusion, when plants are exposed to high temperature, it is speculated that ALA6 may have an effect on the membrane permeability and stability, and this alteration can induce changes in membrane proteins and eventually trigger heat-associated gene expression. However, the physiological functions and underlying mechanisms of these altered genes in ala6 mutants require further study.
Conclusion
ALA6, a P4-type ATPase of Arabidopsis, possesses the conserved structure and function of this family and serves as a phospholipid flippase to maintain membrane stability and fluidity under normal or adverse conditions. The seedlings of loss-of-function mutant ala6 were found to wither, turn yellow and eventually die with exposure to basal thermotolerance or acquired thermotolerance treatments. Overexpression of ALA6 can rescue this phenotype, while transgenic plants containing a point-mutation in a conserved region of ALA6 show no response to heat stress. This suggests that ALA6 is significant for response to high temperature stress. Moreover, ala6 seedlings exhibit higher ion-leakage and lower chlorophyll content after heat treatment, indicating that ALA6 can protect membranes from the disordered state that results from heat stress. This protective effect involves the maintenance of membrane permeability, stability and integrity. In addition, transcriptome analysis shows that ALA6 affects the expression of heat-inducible genes. Taken together, these evidences show that ALA6 plays an essential role in cellular responses to high temperature. In addition, characterization of ALA6 activity and its roles should further illuminate the mechanisms of the involvement of P4-ATPase in stress responses.
Accession Number
RNA sequencing data is available at the National Center for Biotechnology Information (NCBI) data repository (accession PRJNA390831).
Author Contributions
YN and YX designed research; YN, DQ, BL, JM, DW, XW, and WH performed research; YN, DQ, BL, JM analyzed data; and YN, DQ, and YX wrote the paper.
Funding
This work was supported by the National Natural Science Foundation of China (31400220) and the Fundamental Research Funds for the Central Universities (lzujbky-2014-92, lzujbky-2017-156, lzujbky-2017-k14).
Abbreviations
AGP, Arabinogalactan protein; AOC, Allene oxide cyclase; BBX, B-box domain protein; Chl, Chlorophyll; DEG, Differentially expressed gene; EL, Electrolyte leakage; ERF, Ethylene response factor; FPKM, Fragments per kilobase of transcript per million fragments mapped; GUS, β-Glucuronidase; HSP, Heat shock protein; JA, Jasmonic acid; MGDG, Monogalactosyldiacylglycerol; PC, Phosphatidylcholine; PCR, Polymerase chain reaction; PE, Phosphatidylethanolamine; PS, Phosphatidylserine; PSII, Photosystem II; SLAH, Slow anion channel-associated homolog; UBQ, Ubiquitin; WRKY, Transcription factor with conserved amino acid sequence WRKYGQK.
Conflict of Interest Statement
The authors declare that the research was conducted in the absence of any commercial or financial relationships that could be construed as a potential conflict of interest.
Supplementary Material
The Supplementary Material for this article can be found online at: http://journal.frontiersin.org/article/10.3389/fpls.2017.01732/full#supplementary-material
Footnotes
References
Alder-Baerens, N., Lisman, Q., Luong, L., Pomorski, T., and Holthuis, J. C. (2006). Loss of P4 ATPases Drs2p and Dnf3p disrupts aminophospholipid transport and asymmetry in yeast post-Golgi secretory vesicles. Mol. Biol. Cell 17, 1632–1642. doi: 10.1091/mbc.E05-10-0912
Alonso, J. M., Stepanova, A. N., Leisse, T. J., Kim, C. J., Chen, H., Shinn, P., et al. (2003). Genome-wide insertional mutagenesis of Arabidopsis thaliana. Science 301, 653–657. doi: 10.1126/science.1086391
Andersen, J. P., Vestergaard, A. L., Mikkelsen, S. A., Mogensen, L. S., Chalat, M., and Molday, R. S. (2016). P4-ATPases as phospholipid flippases-structure, function, and enigmas. Front. Physiol. 7:275. doi: 10.3389/fphys.2016.00275
Axelsen, K. B., and Palmgren, M. G. (1998). Evolution of substrate specificities in the P-type ATPase superfamily. J. Mol. Evol. 46, 84–101. doi: 10.1007/PL00006286
Axelsen, K. B., and Palmgren, M. G. (2001). Inventory of the superfamily of P-type ion pumps in Arabidopsis. Plant Physiol. 126, 696–706.
Baldridge, R. D., Xu, P., and Graham, T. R. (2013). Type IV P-type ATPases distinguish mono-versus diacyl phosphatidylserine using a cytofacial exit gate in the membrane domain. J. Biol. Chem. 288, 19516–19527. doi: 10.1074/jbc.M113.476911
Banti, V., Mafessoni, F., Loreti, E., Alpi, A., and Perata, P. (2010). The heat-inducible transcription factor HsfA2 enhances anoxia tolerance in Arabidopsis. Plant Physiol. 152, 1471–1483. doi: 10.1104/pp.109.149815
Bi, A., Fan, J., Hu, Z., Wang, G., Amombo, E., Fu, J., et al. (2016). Differential acclimation of enzymatic antioxidant metabolism and photosystem II photochemistry in tall fescue under drought and heat and the combined stresses. Front. Plant Sci. 7:453. doi: 10.3389/fpls.2016.00453
Botella, C., Sautron, E., Boudiere, L., Michaud, M., Dubots, E., Yamaryo-Botté, Y., et al. (2016). ALA10, a phospholipid flippase, controls FAD2/FAD3 desaturation of phosphatidylcholine in the ER and affects chloroplast lipid composition in Arabidopsis thaliana. Plant Physiol. 170, 1300–1314. doi: 10.1104/pp.15.01557
Boudière, L., Michaud, M., Petroutsos, D., Rébeillé, F., Falconet, D., Bastien, O., et al. (2014). Glycerolipids in photosynthesis: composition, synthesis and trafficking. Biochim. Biophys. Acta 1837, 470–480. doi: 10.1016/j.bbabio.2013.09.007
Carratù, L., Franceschelli, S., Pardini, C. L., Kobayashi, G. S., Horvath, I., Vigh, L., et al. (1996). Membrane lipid perturbation modifies the set point of the temperature of heat shock response in yeast. Proc. Natl. Acad. Sci. U.S.A. 93, 3870–3875.
Cazalé, A. C., Clément, M., Chiarenza, S., Roncato, M. A., Pochon, N., Creff, A., et al. (2009). Altered expression of cytosolic/nuclear HSC70-1 molecular chaperone affects development and abiotic stress tolerance in Arabidopsis thaliana. J. Exp. Bot. 60, 2653–2664. doi: 10.1093/jxb/erp109
Clarke, S. M., Cristescu, S. M., Miersch, O., Harren, F. J., Wasternack, C., and Mur, L. A. (2009). Jasmonates act with salicylic acid to confer basal thermotolerance in Arabidopsis thaliana. New Phytol. 182, 175–187. doi: 10.1111/j.1469-8137.2008.02735.x
Clough, S. J., and Bent, A. F. (1998). Floral dip: a simplified method for Agrobacterium-mediated transformation of Arabidopsis thaliana. Plant J. 16, 735–743. doi: 10.1046/j.1365-313x.1998.00343.x
Coleman, J. A., Kwok, M. C., and Molday, R. S. (2009). Localization, purification, and functional reconstitution of the P4-ATPase Atp8a2, a phosphatidylserine flippase in photoreceptor disc membranes. J. Biol. Chem. 284, 32670–32679. doi: 10.1074/jbc.M109.047415
Coleman, J. A., Quazi, F., and Molday, R. S. (2013). Mammalian P4-ATPases and ABC transporters and their role in phospholipid transport. Biochim. Biophys. Acta 1831, 555–574. doi: 10.1016/j.bbalip.2012.10.006
Demidchik, V., Straltsova, D., Medvedev, S. S., Pozhvanov, G. A., Sokolik, A., and Yurin, V. (2014). Stress-induced electrolyte leakage: the role of K+-permeable channels and involvement in programmed cell death and metabolic adjustment. J. Exp. Bot. 65, 1259–1270. doi: 10.1093/jxb/eru004
Ding, J., Wu, Z., Crider, B. P., Ma, Y., Li, X., Slaughter, C., et al. (2000). Identification and functional expression of four isoforms of ATPase II, the putative aminophospholipid translocase. Effect of isoform variation on the ATPase activity and phospholipid specificity. J. Biol. Chem. 275, 23378–23386. doi: 10.1074/jbc.M910319199
Fragkostefanakis, S., Röth, S., Schleiff, E., and Scharf, K. D. (2015). Prospects of engineering thermotolerance in crops through modulation of heat stress transcription factor and heat shock protein networks. Plant Cell Environ. 38, 1881–1895. doi: 10.1111/pce.12396
Gangappa, S. N., and Botto, J. F. (2014). The BBX family of plant transcription factors. Trends Plant Sci. 19, 460–470. doi: 10.1016/j.tplants.2014.01.010
Gaspar, Y. M., Nam, J., Schultz, C. J., Lee, L. Y., Gilson, P. R., Gelvin, S. B., et al. (2004). Characterization of the Arabidopsis lysine-rich arabinogalactan-protein AtAGP17 mutant(rat1) that results in a decreased efficiency of agrobacterium transformation. Plant Physiol. 135, 2162–2171.
Gomès, E., Jakobsen, M. K., Axelsen, K. B., Geisler, M., and Palmgren, M. G. (2000). Chilling tolerance in Arabidopsis involves ALA1, a member of a new family of putative aminophospholipid translocases. Plant Cell 12, 2441–2454.
Guo, Z., Lu, J., Wang, X., Zhan, B., Li, W., and Ding, S. W. (2017). Lipid flippases promote antiviral silencing and the biogenesis of viral and host siRNAs in Arabidopsis. Proc. Natl. Acad. Sci. U.S.A. 114, 1377–1382. doi: 10.1073/pnas.1614204114
Horváth, I., Glatz, A., Nakamoto, H., Mishkind, M. L., Munnik, T., Saidi, Y., et al. (2012). Heat shock response in photosynthetic organisms: membrane and lipid connections. Prog. Lipid Res. 51, 208–220. doi: 10.1016/j.plipres.2012.02.002
Horváth, I., Glatz, A., Varvasovszki, V., Török, Z., Páli, T., Balogh, G., et al. (1998). Membrane physical state controls the signaling mechanism of the heat shock response in Synechocystis PCC 6803: identification of hsp17 as a “fluidity gene”. Proc. Natl. Acad. Sci. U.S.A. 95, 3513–3518.
Jefferson, R. A., Kavanagh, T. A., and Bevan, M. W. (1987). GUS fusions: beta-glucuronidase as a sensitive and versatile gene fusion marker in higher plants. EMBO J. 6, 3901–3907.
Larkindale, J., and Vierling, E. (2008). Core genome responses involved in acclimation to high temperature. Plant Physiol. 146, 748–761.
Lopez-Marques, R. L., Poulsen, L. R., Bailly, A., Geisler, M., Pomorski, T. G., and Palmgren, M. G. (2014). Structure and mechanism of ATP-dependent phospholipid transporters. Biochim. Biophys. Acta 1850, 461–475. doi: 10.1016/j.bbagen.2014.04.008
López-Marqués, R. L., Poulsen, L. R., Hanisch, S., Meffert, K., Buch-Pedersen, M. J., Jakobsen, M. K., et al. (2010). Intracellular targeting signals and lipid specificity determinants of the ALA/ALIS P4-ATPase complex reside in the catalytic ALA alpha-subunit. Mol. Biol. Cell 21, 791–801. doi: 10.1091/mbc.E09-08-0656
McDowell, S. C., López-Marqués, R. L., Cohen, T., Brown, E., Rosenberg, A., Palmgren, M. G., et al. (2015). Loss of the Arabidopsis thaliana P4-ATPases ALA6 and ALA7 impairs pollen fitness and alters the pollen tube plasma membrane. Front. Plant Sci. 6:197. doi: 10.3389/fpls.2015.00197
McDowell, S. C., López-Marqués, R. L., Poulsen, L. R., Palmgren, M. G., and Harper, J. F. (2013). Loss of the Arabidopsis thaliana P4-ATPase ALA3 reduces adaptability to temperature stresses and impairs vegetative, pollen, and ovule development. PLOS ONE 8:e62577. doi: 10.1371/journal.pone.0062577
Mittler, R., Finka, A., and Goloubinoff, P. (2012). How do plants feel the heat? Trends Biochem. Sci. 37, 118–125. doi: 10.1016/j.tibs.2011.11.007
Murata, N., and Los, D. A. (1997). Membrane fluidity and temperature perception. Plant Physiol. 115, 875–879.
Nieto-Sotelo, J., Martínez, L. M., Ponce, G., Cassab, G. I, Alagón, A., Meeley, R. B., et al. (2002). MaizeHSP101plays important roles in both induced and basal thermotolerance and primary root growth. Plant Cell 14, 1621–1633.
Palmgren, M. G., and Axelsen, K. B. (1998). Evolution of P-type ATPases. Biochim. Biophys. Acta 1365, 37–45. doi: 10.1016/S0005-2728(98)00041-3
Paterson, J. K., Renkema, K., Burden, L., Halleck, M. S., Schlegel, R. A., Williamson, P., et al. (2006). Lipid specific activation of the murine P4-ATPase Atp8a1(ATPaseII). Biochemistry 45, 5367–5376. doi: 10.1021/bi052359b
Paulusma, C. C., and Elferink, R. P. (2010). P4 ATPases-the physiological relevance of lipid flipping transporters. FEBS Lett. 584, 2708–2716. doi: 10.1016/j.febslet.2010.04.071
Pedersen, P. L., and Carafoli, E. (1987). Ion motive ATPases I: ubiquity, properties and significance to cell function. Trends Biochem. Sci. 12, 146–150. doi: 10.1016/0968-0004(87)90071-5
Pomorski, T., Lombardi, R., Riezman, H., Devaux, P. F., van Meer, G., and Holthuis, J. C. M. (2003). Drs2p related P-type ATPases Dnf1p and Dnf2p are required for phospholipid translocation across the yeast plasma membrane and serve a role in endocytosis. Mol. Biol. Cell 14, 1240–1254. doi: 10.1091/mbc.E02-08-0501
Pomorski, T. G., and Menon, A. K. (2006). Lipid flippases and their biological functions. Cell. Mol. Life Sci. 63, 2908–2921. doi: 10.1007/s00018-006-6167-7
Pomorski, T. G., and Menon, A. K. (2016). Lipid somersaults: uncovering the mechanisms of protein-mediated lipid flipping. Prog. Lipid. Res. 64, 69–84. doi: 10.1016/j.plipres.2016.08.003
Poulsen, L. R., López-Marqués, R. L., McDowell, S. C., Okkeri, J., Licht, D., Schulz, A., et al. (2008). The Arabidopsis P4-ATPase ALA3 localizes to the Golgi and requires a beta-subunit to function in lipid translocation and secretory vesicle formation. Plant Cell 20, 658–676. doi: 10.1105/tpc.107.054767
Poulsen, L. R., López-Marqués, R. L., Pedas, P. R., McDowell, S. C., Brown, E., Kunze, R., et al. (2015). A phospholipid uptake system in the model plant Arabidopsis thaliana. Nat. Commun. 27, 7649. doi: 10.1038/ncomms8649
Puts, C. F., and Holthuis, J. C. (2009). Mechanism and significance of P4 ATPase-catalyzed lipid transport: lessons from a Na+/K+-pump. Biochim. Biophys. Acta. 1791, 603–611. doi: 10.1016/j.bbalip.2009.02.005
Riekhof, W. R., and Voelker, D. R. (2006). Uptake and utilization of lyso-phosphatidylethanolamine by Saccharomyces cerevisiae. J. Biol. Chem. 281, 36588–36596. doi: 10.1074/jbc.M608851200
Rieu, I., and Powers, S. J. (2009). Real-time quantitative RT-pcr: design, calculations, and statistics. Plant Cell 21, 1031–1033. doi: 10.1105/tpc.109.066001
Saidi, Y., Finka, A., and Goloubinoff, P. (2011). Heat perception and signalling in plants: a tortuous path to thermotolerance. New Phytol. 190, 556–565. doi: 10.1111/j.1469-8137.2010.03571.x
Sairam, R. K., and Srivastava, G. C. (2002). Changes in antioxidant activity in subcellular fraction of tolerant and susceptible wheat genotypes in response to long term salt stress. Plant Sci. 162, 897–904. doi: 10.1016/S0168-9452(02)00037-7
Sebastian, T. T., Baldridge, R. D., Xu, P., and Graham, T. R. (2012). Phospholipid flippases: building asymmetric membranes and transport vesicles. Biochim. Biophys. Acta 1821, 1068–1077. doi: 10.1016/j.bbalip.2011.12.007
Stenzel, I., Otto, M., Delker, C., Kirmse, N., Schmidt, D., Miersch, O., et al. (2012). ALLENE OXIDE CYCLASE (AOC) gene family members of Arabidopsis thaliana: tissue- and organ-specific promoter activities and in vivo heteromerization. J. Exp. Bot. 63, 6125–6138. doi: 10.1093/jxb/ers261
Van Der Mark, V. A., Elferink, R. P., and Paulusma, C. C. (2013). P4 ATPases: flippases in health and disease. Int. J. Mol. Sci. 14, 7897–7922. doi: 10.3390/ijms14047897
Vigh, L., Escribá, P. V., Sonnleitner, A., Sonnleitner, M., Piotto, S., Maresca, B., et al. (2005). The significance of lipid composition for membrane activity: new concepts and ways of assessing function. Prog. Lipid Res. 44, 303–344. doi: 10.1016/j.plipres.2005.08.001
Vigh, L., Maresca, B., and Harwood, J. (1998). Does the membrane’s physical state control the expression of heat shock and other genes? Trends Biochem. Sci. 23, 369–374. doi: 10.1016/S0968-0004(98)01279-1
Vigh, L., Nakamoto, H., Landry, J., Gomez-Munoz, A., Harwood, J. L., and Horvath, I. (2007). Membrane regulation of the stress response from prokaryotic models to mammalian cells. Ann. N. Y. Acad. Sci. 1113, 40–51. doi: 10.1196/annals.1391.027
Wang, Q., Tu, X., Zhang, J., Chen, X., and Rao, L. (2013). Heat stress-induced BBX18 negatively regulates the thermotolerance in Arabidopsis. Mol. Biol. Rep. 40, 2679–2688. doi: 10.1007/s11033-012-2354-9
Woo, H. R., Chung, K. M., Park, J. H., Oh, S. A., Ahn, T., Hong, S. H., et al. (2001). ORE9, an F-box protein that regulates leaf senescence in Arabidopsis. Plant Cell 13, 1779–1790. doi: 10.1105/TPC.010061
Xu, P., Okkeri, J., Hanisch, S., Hu, R. Y., Xu, Q., Pomorski, T. G., et al. (2009). Identification of a novel mouse P4-ATPase family member highly expressed during spermatogenesis. J. Cell Sci. 122, 2866–2876. doi: 10.1242/jcs.047423
Yu, J., Cheng, Y., Feng, K., Ruan, M., Ye, Q., Wang, R., et al. (2016). Genome-wide identification and expression profiling of tomatoHsp20gene family in response to biotic and abiotic stresses. Front. Plant Sci. 7:1215. doi: 10.3389/fpls.2016.01215
Zhang, A., Ren, H. M., Tan, Y. Q., Qi, G. N., Yao, F. Y., Wu, G. L., et al. (2016). S-type anion channels SLAC1 and SLAH3 function as essential negative regulators of inward K+ channels and stomatal opening in Arabidopsis. Plant Cell 28, 949–965. doi: 10.1105/tpc.15.01050
Zhang, X., Wollenweber, B., Jiang, D., Liu, F., and Zhao, J. (2008). Water deficits and heat shock effects on photosynthesis of a transgenic Arabidopsis thaliana constitutively expressing ABP9, a bZIP transcription factor. J. Exp. Bot. 59, 839–848. doi: 10.1093/jxb/erm364
Keywords: Arabidopsis P4-type ATPase, Aminophospholipid ATPase6 (ALA6), ALA6 T-DNA insertion mutant (ala6), lipid flippase, membrane, heat stress response
Citation: Niu Y, Qian D, Liu B, Ma J, Wan D, Wang X, He W and Xiang Y (2017) ALA6, a P4-type ATPase, Is Involved in Heat Stress Responses in Arabidopsis thaliana. Front. Plant Sci. 8:1732. doi: 10.3389/fpls.2017.01732
Received: 03 May 2017; Accepted: 21 September 2017;
Published: 04 October 2017.
Edited by:
Yi Ma, University of Connecticut, United StatesReviewed by:
Shengcheng Han, Beijing Normal University, ChinaJoshua Blakeslee, The Ohio State University, United States
Copyright © 2017 Niu, Qian, Liu, Ma, Wan, Wang, He and Xiang. This is an open-access article distributed under the terms of the Creative Commons Attribution License (CC BY). The use, distribution or reproduction in other forums is permitted, provided the original author(s) or licensor are credited and that the original publication in this journal is cited, in accordance with accepted academic practice. No use, distribution or reproduction is permitted which does not comply with these terms.
*Correspondence: Yue Niu, niuy@lzu.edu.cn Yun Xiang, xiangy@lzu.edu.cn